Rydberg
spectroscopy and dynamics
Douglas Lazenby, Dr Russell Minns
Rydberg states
are highly excited electronic states in which one electron has been
promoted to a high lying electronic state (typically with principal
quantum number n > 8). These highly excited states have some
remarkable properties, for example, the spatial extent of a Rydberg
state scales as the square of the principal quantum number n, so for n = 10, the inner and outer turning points
are around 10 nm apart, and for n = 30, they are almost one tenth
of a micron apart, which is nearly macroscopic. Another interesting
feature is the period of electronic motion. A Rydberg electron with
n = 30 will have a classical orbit period of almost 5 ps, which
is of the same order of magnitude as the period of molecular rotation
in a small molecule, and is around one thousand times slower than
a typical molecular vibration. Consequently the Born-Oppenheimer
approximation breaks down.
Wave packets are spatially localised quantum states possessing both
particle-like and wave-like characteristics. They provide an important
bridge between quantum mechanics and the classical picture of a
particle moving on a classical trajectory. Electronic wave packets
created from coherent superpositions of Rydberg states are particularly
attractive. Rydberg wave packets in atoms have been the focus of
a great deal of interest during the last two decades. Initially,
the impetus was on testing the correspondence principle limit of
an atom, as invoked by Neils Bohr; although currently, as a result
of huge technological advances in ultrafast laser technology, there
has been a shift in emphasis from the observation of Rydberg wave
packets to their customisation and control.
Molecular
Rydberg wave packets are even more attractive because of the additional
degrees of freedom introduced by the molecular core. These systems
exhibit many of the dynamical complications associated with larger
molecules of more interest to chemists and biologists, such as the
high density of rovibronic states and non-adiabatic coupling, yet
they remain highly tractable.
In
our group we employ frequency-resolved and time-resolved spectroscopy
techniques to investigate Rydberg dynamics in atoms and diatomic
molecules, both experimentally and theoretically. One of our aims
is to take these highly tractable molecular Rydberg systems and
design laser pulse sequences or laser pulse shapes to control the
non-adiabatic dynamics. Within the last few years we have employed
sequences of phase-locked optical pulses to control the orbital
angular momentum composition of Rydberg wave packets in Xe and Na,
and the rotational angular momentum of Rydberg wave packets in NO.
We have also made progress in controlling the autoionisation/predissociation
branching ratio in NO. To complement and guide the dynamics experiments
we are also interested in the high resolution spectroscopy of highly
excited Rydberg states and have recently reported the spectroscopy
of Rydberg states of NO in external dc electric fields.
As well as using optical pulses to control Rydberg dynamics, we are interested in investigating how DC electric fields can be exploited as control tools. We have recently reported the first observation of the important role that slew rate plays in the field-ionisation of molecular Rydberg species, and demonstrated its potential for controlling the rotational quantum state of the product ions.
In collaboration with Professor Christian Jungen (Orsay) we have developed a time-dependent extension of multichannel quantum theory (MQDT) in which time-dependent wave packets are constructed using first-order perturbation theory. We have derived an analytical expression for the complex excitation function for a sequence of excitation pulses and have developed numerical methods to model the complex excitation function for phase and amplitude shaped excitation pulses. Our ultimate goal is to design rational light fields to control the branching ratio of ionisation/dissociation.
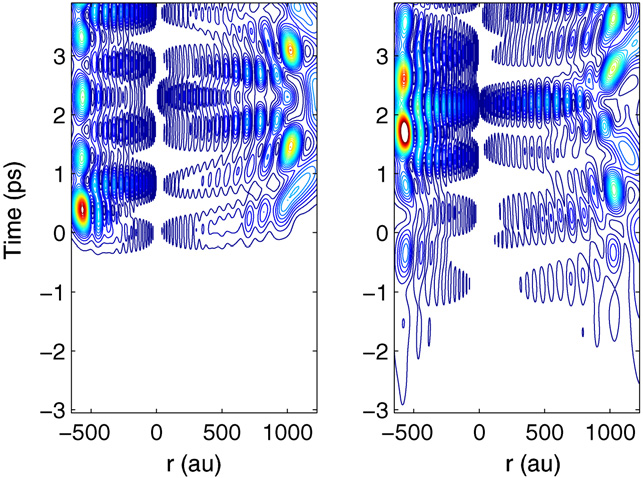
Above: Excitation of a Rydberg electron wave packet in H2 below the lowest ionisation limit. Comparison of the excitation process with the unshaped reference pulse A (left panel) and a phase-shaped pulse B (right panel). The contour plot shows the wave packet probability density as a function of radial distance r (au) and time t (ps). Negative radial distances r correspond to the dynamics in channel j = 2 (ion core rotational quantum number N+ = 2), while positive radial distances correspond to channel j = 1 (N+ = 0). In both channels, the wave packet oscillates between the core (r ≈ 0) and the outer turning point. For pulse A (left panel), the excitation at time t ≈ 0 ps is almost instant and is quickly followed by free evolution of the wave packet. For pulse B (right panel), the excitation process with the shaped pulse begins already at times t < −5 ps, becomes discernible in the contour plot at times t ≈−3 ps and does not evolve freely until for times t > 5 ps. See J. Phys. B. 41 074022 (2008) for details.
Back
to Top
Organic
photochemistry
Roman Spesyvtsev, Dr Russell Minns
Chemists have
long dreamt of using laser light to control chemical reactivity;
but unfortunately, it is not possible to break a specific bond in
a molecule simply by tuning a laser into resonance with a transition
to that bond. This is because the energy put into the molecule is
redistributed very rapidly on the timescale of molecular vibrations.
However, since the advent of femtosecond lasers (1 fs = 0.000 000
000 000 001 s) in the 1980s it has become possible to probe chemical
bonds as they are being made and broken and, with further advances
in laser technology in the late 1990s, it is now possible to generate
complex femtosecond laser pulses that can control these processes.
The most common approach to controlling molecular bond breaking
has been to employ a learning algorithm and a feedback loop from
which the laser optimises its own pulse shape. Such ‘black
box’ techniques have been exploited with huge success tocontrol
bond dissociation in organometallic complexes. Obviously a great
deal of information about the dynamics is concealed within these
complex laser pulses but, to date, it has proved difficult to unravel
this information.
In collaboration
with Professor
Mike Robb (Imperial), Dr
Mike Bearpark (Imperial) and Dr
Graham Worth (Birmingham), we are developing the machinery to
investigate the dynamics of photochemical reactions in fine detail. Once we have a detailed understanding our aim is to employ shaped light fields to control the dynamics on the excited potential energy surface, and hence control when the molecule returns to the ground state.
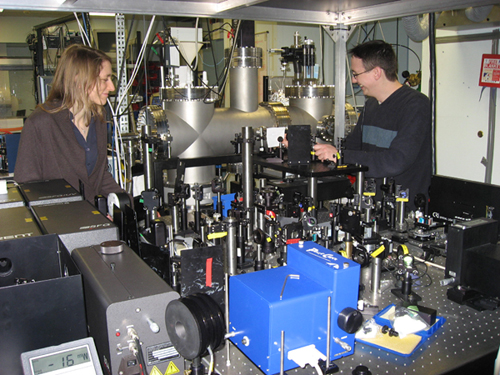
Above: Photoelectron
imaging vacuum apparatus and uv-femtosecond
pulse shaping and characterisation setup.
We have recently investigated the ultrafast intramolecular dynamics of electronically and vibrationally excited benzene using time-resolved photoelectron spectroscopy and quantum dynamics simulations. In addition to an ultrafast initial decay we observe an oscillation between two states. The most plausible explanation of the data is that the photophysical pathway involves an ultrafast intersystem crossing from the initially populated singlet state to an optically dark triplet state. Our results challenge the currently accepted view that the initial ultrafast decay from the excited state is dominated by internal conversion through a singlet-singlet conical intersection taking the population directly back to the ground state.
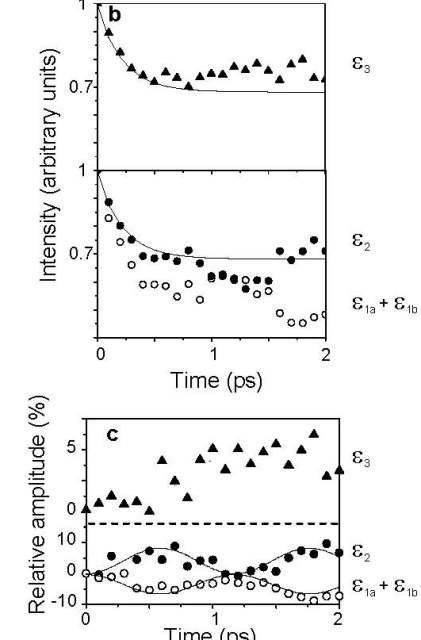
Above: Time-resolved dynamics of benzene during intersystem crossing between S1 and T2 and the subsequent internal conversion to T1. Curves e1a and e1b correspond to photoelectron energies associated with ionisation from S1 and are separated by 0.1 eV, which corresponds to the v1 (a1g) breathing mode in the benzene cation. e2 corresponds to photoelectron energies associated with ionisation from T2 and e3 corresponds to photoelectron energies associated with ionisation from T1. (b) Integrated intensities of photoelectrons as a function of pump-probe delay. The open circles correspond to integration over the range e1a = 1.25-2.35 eV and e1b = 1.13-1.23 eV, and the closed circles correspond to integration over the range e2 = 0.75-0.95 eV. The filled triangles correspond to integration over the range e3 = 0.35-0.45 eV. (c) The same data as (b) but with the exponential decay removed. The solid lines in the lower half of the graph are a least squares sinusoidal fit to the data and have periods 1.2 ps. See Chem. Phys. Lett. 469 43-47 (2009) for details.
We have also developed a robust method for producing tuneable, shaped, UV femtosecond laser pulses by shaping and frequency doubling the output of a commercial optical parametric amplifier (OPA). A reflective mode, folded, pulse shaping assembly employing a spatial light modulator (SLM) shapes femtosecond pulses in the visible region of the spectrum. The shaped visible light pulses are frequency doubled to generate phase- and amplitude-shaped, ultrashort light pulses in the deep UV. This approach benefits from a simple experimental setup and the potential for tuning the central frequency of the shaped UV waveform. A number of pulse shapes have been synthesised and characterised using cross-correlation frequency resolved optical gating (XFROG), including pairs of pulses with different central wavelengths.
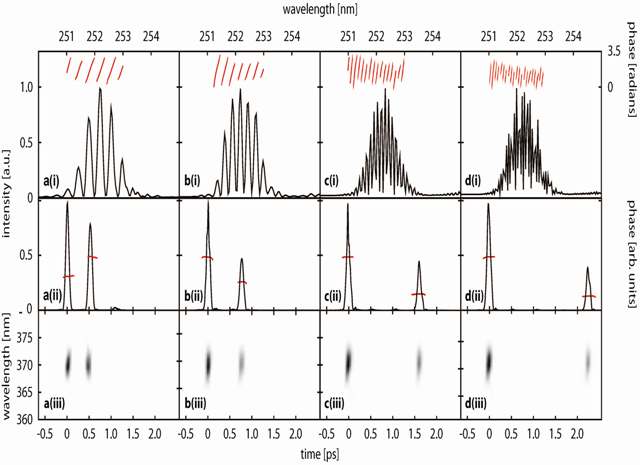
Above: Pairs of pulses in the time domain generated from combs of alternating 0 and p phase. Each figure shows a raw XFROG trace (iii), the reconstructed temporal electric field intensity of the UV pulse (black) and phase (red)(ii) and reconstructed spectral intensity (black) and phase (red) (i) of the UV pulse. See Appl. Phys. B - Lasers and Optics 94 181-186 (2009) for details.
We are just beginning a new project, in collaboration with Dr Jonathan Underwood, to combine our methods with molecular axis alignment to gain further insight into the dynamics and for developing potential control schemes. This project is funded by the EU Marie-Curie Initial Training Network FASTQUAST.
Back
to Top
Chromophores in
photobiology
Ciaran Mooney
Understanding ultrafast processes is of great importance in elucidating protein dynamics. For many biological targets the elementary aspects of these processes are still not well understood. Fluorescent proteins are a good example of this: they are used widely in cell biology, primarily in fluorescence microscopy, yet there are still gaps in our understanding of the fundamental photochemistry and photophysics which can lead to significant pitfalls in applications, ranging from artefacts in measurements to cell death. In contrast to isolated gas-phase systems, the environment of the chromophore in a fluorescent protein plays an essential role in determining the reaction pathway and product distribution. In solution based reactions the solvent molecules move around freely within the solute, whereas in protein based reactions the protein environment provides both a static and dynamical constraint on the motions of the atoms within the chromophore. The protein environment effectively catalyses a photoreaction path that may not be observed in solution at all, but which may be crucial for a specific biological function.
In collaboration
with Dr Adam McKay and Professor
Steve Caddick we are developing a novel experiment to investigate
the femtosecond photoinduced dynamics of large proteins, based on
a combination of electrospray ionisation mass-spectrometry and time-resolved
photoelectron imaging.
Our instrument is currently being commissioned and our progress is logged below.
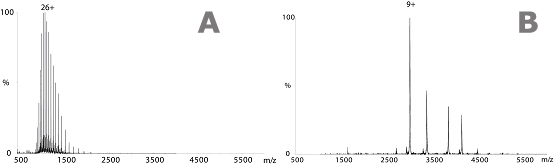
Above: (A) Mass spectrum obtained under denaturing conditions. Protein mass spectrometry experiments used to confirm molecular weights are typically carried out in acidified aqueous/organic solvent solutions. This produces numerous highly charged protein ions and accurate molecular weights, however, the proteins are denatured and all structural information is lost. (B) Mass spectrum of GFP obtained under ‘native-like’ conditions. The use of aqueous buffers such as ammonium acetate allows the protein to be transferred into the gas-phase in a ‘native-like’ state.
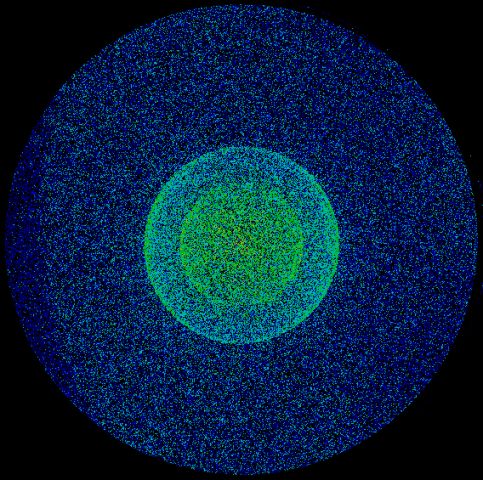
Above: Anions are imaged onto an MCP detector coupled to a phosphore screen and CCD camera (left image). Anions from the pulsed electrospray source are focussed into the source region of a velocity map imaging setup and the two rings in the image on the right arise from photodetachment of the outer electron from I- anions which are being used to calibrate the apparatus.
Back
to Top
Ultrafast
photochemistry on metal surfaces
Jadranka Butorac
Surface photochemical
processes are an important class of photoreactions, both from a
fundamental point of view and also in practical terms. For example,
improving our understanding of photon-induced surface processes
is a key factor in the design of industrial and environmental catalysts
and in the understanding of surface reactions in the atmosphere
and in space. Femtosecond laser-induced photochemistry on metal
surfaces involves an initial optical excitation of electrons in
the metal. This excitation leads to a highly non-equilibrium situation
in which the electrons, often referred to as ‘hot’ electrons,
have a temperature of several thousand degrees Kelvin. In contrast,
the phonon bath within the solid remains relatively cool. On a femtosecond
timescale, these hot electrons attach to unoccupied energy levels
of the adsorbate. The nuclei are no longer in their equilibrium
positions and the adsorbate vibrates, resulting in subsequent desorption
or reaction of the adsorbed species. Meanwhile, on a picosecond
timescale, the hot electron distribution equilibrates with the lattice
and the heated phonon bath can then itself induce desorption and
reaction by thermal activation.
In collaboration
with Dr
Wendy Brown we have designed a new experiment to follow the
various processes in laser-induced surface reactions in real time,
with the ultimate goal of being able to understand and control surface
photochemistry.
Our experiments
employ a novel combination of two-photon correlation (2PC) and time-resolved
photoelectron spectroscopy (TRPES). The 2PC technique allows us
to determine which reactions are electron and phonon mediated, and
to measure the timescale of the reaction processes. In these experiments,
the photoreaction yield is measured as a function of the delay time
between two, equally intense, laser pulses. Because of the fast
coupling between the hot electrons and the phonons, high electronic
temperatures result from the combined effect of the two laser pulses,
provided that they are separated by a time delay less than the electron-phonon
equilibration time of ~1 ps. Hence, a fast response is observed
for electron mediated processes. Alternatively, the observation
of a slow response implies a phonon mediated process, as the phonon
cooling time is ~50 ps.
TRPES is used
to monitor the real time changes in the electronic structure of
the adsorbed reactants and products. For the TRPES experiments a
pump pulse, identical to that used in the 2PC experiments, initiates
the photochemical reaction. A delayed vacuum ultraviolet (VUV) femtosecond
probe pulse will then subsequently ionise the substrate-surface
system and the resulting photoelectron spectrum will provide a fingerprint
of the valence energy level electronic structure. The probe pulse
is a single harmonic of the fundamental generated in a high harmonic
generation (HHG) capillary cell, separated from the fundamental
and other harmonics using a combination of foils and multilayer
reflectors. The kinetic energies of all the photoemitted electrons
are measured simultaneously using a commercial hemispherical electron
analyser. Our first experiment is to monitor the photo-initiated reaction
of CO and NO on a Pd(111) surface. We will then investigate the
possibility of coherent control of this reaction using phase shaped
infrared femtosecond laser pulses.
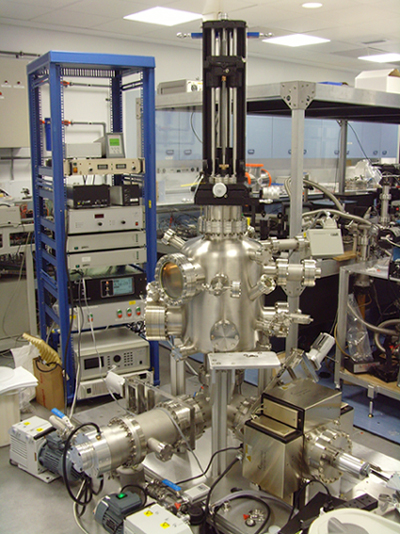
Above: Ultrahigh
vacuum chamber for time-resolved surface photochemistry. The chamber
is equipped for traditional surface science techniques: reflection
absorption infra-red spectroscopy, temperature programmed
desorption, low energy electron diffraction, auger
electron spectroscopy. It also has a hemispherical analyser for time-resolved photoelectron spectroscopy. It will soon be coupled with our source of femtosecond VUV pulses being created by high harmonic generation in a capillary of Ar.
Back
to Top
Attosecond
science
All fundamental
processes in chemistry, biology and materials science involve the
motions of electrons and the nuclei of the constituent atoms. The
extremely fast electronic motions have attosecond timescales (1
attosecond = 1 thousandth of a femtosecond). A proper understanding
of the way in which electronic motions influence these fundamental
processes requires probes on the attosecond timescale. We are interested
in exploiting attosecond light sources to investigate organic photochemical
reactions. This project is carried out in collaboration with Professor
Jon Marangos and Dr
John Tisch at Imperial College.
Back
to Top
This page last modified
14 November, 2009
by Helen Fielding
|